Biological Elements of Psychopathology
Learning Objectives
- Explain polygenic inheritance and genetic heritability in mental disorders
- Explain neural communication and the role of neurotransmitters in neural transmission
Biological Contributions to Mental Disorders
As discussed on the previous page, biological elements such as genetics, brain structures, the endocrine system, and neurotransmitters all play an important role in the development of mental disorders. As explained by the biopsychosocial model, none of these biological factors, on their own can generate a mental disorder, but combined with environmental, psychological, and social or cultural forces, they interact to shape how we learn, how our brains function, which genes are expressed or turned off, and the biological foundation of a person’s diathesis (or vulnerability) to developing a disorder. Further, because these elements have been heavily studied in recent years, many of the medical or psychiatric treatments that have been developed, including drugs such as Zoloft (sertraline) or Prozac (fluoxetine) and many others, are based on what has been learned regarding these biological systems that contribute to psychopathology. It is important to understand this part of this system in order to better understand human functioning and how medical treatments work.
Genetic Contributions
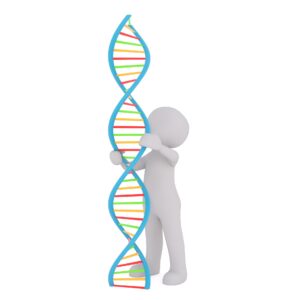
DNA (deoxyribonucleic acid) is present in the nucleus of every cell of our body, packed into larger structures called chromosomes, and shaped in a double-helix (think of a ladder twisted into a spiral shape; see Figure 1). Most human beings have 23 pairs of chromosomes, receiving one set of 23 from our mother and the other set of 23 from our father. The 23rd chromosome, or sex chromosome, differs between males and females. Females have two copies of the X chromosome while males have one X chromosome and one Y chromosome. Our DNA ladder comprises rails made up of sugar and phosphate (blue color in Figure 1), and rungs consisting of four base pairs that are linked together (multicolored, horizontal elements in Figure 1).
Sections of the DNA strand (the rungs of the ladder) containing many of these base pairs make up what we call genes, and they contain the instructions for the construction of proteins. The total of all a person’s genes is called their genome, and the set of genes that may create a specific outcome, such as hair color, is called the genotype (the set of genes that produces something specific). The actual observable result of a genotype is called the phenotype, and it may differ from what is expected from the genotype due to various factors, including environmental interactions, interactions with other genes, epigenetics, and other factors. The Human Genome Project estimates that human beings have between 20,000 and 25,000 genes. Relevant to mental disorders, another concept is very important to remember. There are some rare medical conditions, such as a form of fatal dementia called Huntington’s disease that is caused by a dominant gene. If a person inherits that gene (from either parent), they will develop the disorder. However, this is not true for any of the known mental disorders discussed in this text. All mental disorders have polygenic inheritance; there is no single gene that causes depression, schizophrenia, or anorexia nervosa, for example. Instead, there are multiple (poly) genes that interact to contribute to the development of mental disorders.
Recently, Boyle, Li, and Pritchard, three scientists at Stanford University, proposed an “omnigenic model” hypothesizing that sets of “core genes” may influence most aspects of our lives—from height to the chance of developing schizophrenia—and are each part of large networks of possibly thousands of genes that impact each other. They suggest that core characteristics are not influenced by any singular gene or even group of genes, but by hundreds or thousands of core and periphery genes. As Yong (2017) summarizes, “They’re weak individually, but powerful en masse.“[1]
Another scientist reviewing this omnigenic model, Faraone (2017), gave an example of how this might work in relation to mental disorders: “Let’s posit this sequence of events: 1) in utero, the developing [baby’s] brain is assaulted by a toxic environment or aberrant core genetic variants that pose a risk to survival. 2) As a reaction to this assault, the regulatory mechanisms governed by peripheral genes make adjustments that decrease the risk of death but increase the risk for psychiatric illness. 3) The initial assault may leave a widespread signature in the brain such as reduced brain volumes (as we see for many psychiatric disorders) and 4) The regulatory responses (#2) up- or down-regulate proteins that directly cause psychiatric symptoms.”[2] Thus, it is clear that even a “polygenic” explanation for mental disorders is an overly simplistic statement and as Faraone’s (2017) example illustrates, even a biogenic-focused explanation of mental disorders also involves environmental influences.
Twin Studies and Heritability
In the field of behavioral genetics, the Minnesota Study of Twins Reared Apart—a well-known study of the genetic basis for personality—conducted research with twins from 1979 to 1999. In studying 350 pairs of twins, including pairs of identical and fraternal twins reared together and apart, researchers found that identical twins, whether raised together or apart, have very similar personalities (Bouchard, 1994; Bouchard, Lykken, McGue, Segal, & Tellegen, 1990; Segal, 2012). These findings suggest the heritability of some personality traits. Heritability refers to the proportion of difference among people that is attributed to genetics. In other words, the larger the statistic, the more people will vary on that trait/phenotype due to genetic differences that exist in the population. Some of the traits that the study reported as having more than a 0.50 heritability ratio include leadership, obedience to authority, a sense of well-being, alienation, resistance to stress, and fearfulness. The implication is that some aspects of our personalities are largely influenced by genetics; however, it’s important to point out that traits are not determined by a single gene, but by a combination of many genes, as well as by epigenetic factors that control whether the genes are expressed or not.
Heritability measures how much of the variation of a trait can be attributed to genetic factors, as opposed to environmental factors. Thus, the concept of heritability can be expressed in the form of the following question: “What is the proportion of the variation in a given trait within a population that is not explained by the environment or random chance?” It is calculated by comparing individual variation among related individuals in a population using various methods and is helpful in seeking to understand the impact of genetics versus environmental influences for a given disorder (think back to the comparison of different versions of the biopsychosocial model depending on the disorder in the last module). A related concept is that of concordance, which measures the probability or likelihood that if one person in a pair of people (usually twins) has a specific phenotype, that the other one will also have or will develop that trait.
- The general population has a 1% risk.
- First cousins have 2% risk; they share 12.5% of genes.
- The next relationships are uncles/aunts, nephews/nieces, grandchildren, and half-siblings; they share 25% of genes and the risk ranges from about 3–6%.
- The next relationships are parents, siblings, children, and fraternal twins; they share 50% of genes and the risks are about 6, 9, 13, and 17%, respectively. Identical twins share 100% of genes and have about a 48% risk.
As you go down the graph and review the next slides, you can see that the more genetic overlap there is between two people, the greater the chance that if one of them has schizophrenia, that the other one will also develop the disorder.
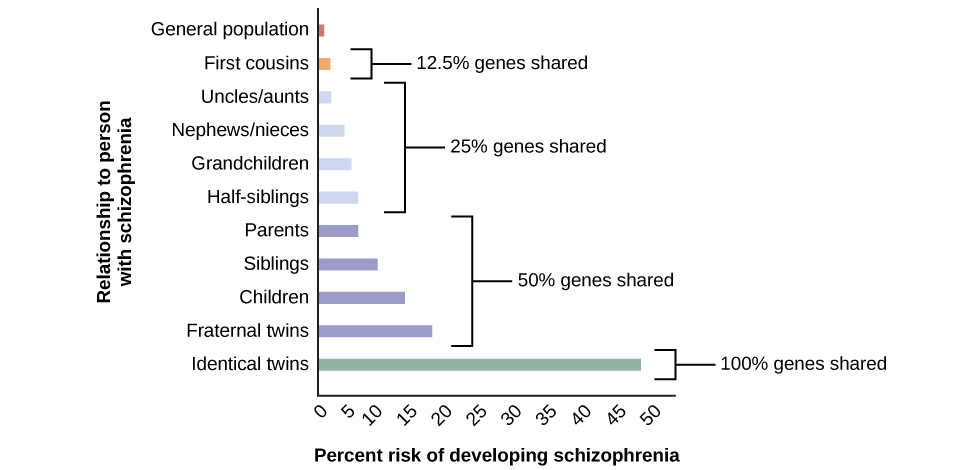
Try It
Neural Transmission—How Neurons Signal Each Other
Those striving to understand the human mind may study the nervous system. Learning how the body’s cells and organs function can help us understand the biological basis of human psychology, which is essential in understanding the biological etiology and treatment of mental disorders. It is especially important to understand how the cells of our brains function; these cells are called neurons.
Neuron Structure
Neurons are the central building blocks of the nervous system, 100 billion strong at birth. Like all cells, neurons consist of several different parts, each serving a specialized function. A neuron’s outer surface is made up of a semipermeable membrane. This membrane allows smaller molecules and molecules without an electrical charge to pass through it while stopping larger or highly charged molecules. (See Figure 3).
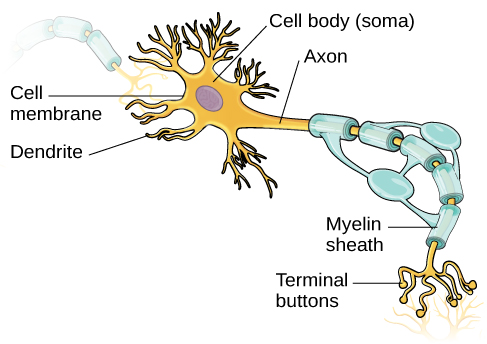
The nucleus of the neuron, where all genetic material is stored and proteins are produced including neurotransmitters, is located in the soma, or cell body. The soma has branching extensions known as dendrites. The neuron is a small information processor, and dendrites serve as input sites where signals are received from other neurons. These signals are transmitted electrically across the soma and down a major extension from the soma known as the axon, which ends at multiple terminal buttons. The terminal buttons contain synaptic vesicles that house neurotransmitters, the chemical messengers of the nervous system.
Axons range in length from a fraction of an inch to several feet. In some axons, glial cells form a fatty substance known as the myelin sheath, which coats the axon and acts as an insulator, increasing the speed at which the signal travels, making neural transmission more efficient. The myelin sheath is crucial for the normal operation of the neurons within the nervous system: the loss of the insulation it provides can be detrimental to normal function. To understand how this works, let’s consider an example. PKU, a genetic disorder, causes a reduction in myelin and abnormalities in white matter of cortical and subcortical structures. The disorder is associated with a variety of conditions including severe cognitive deficits, exaggerated reflexes, and seizures (Anderson & Leuzzi, 2010; Huttenlocher, 2000). Another disorder, multiple sclerosis (MS), an autoimmune disorder, involves a large-scale loss of the myelin sheath on axons throughout the nervous system. The resulting interference in the electrical signal prevents the quick transmittal of information by neurons and can lead to a number of symptoms, such as dizziness, fatigue, loss of motor control, and sexual dysfunction. While some treatments may help to modify the course of the disease and manage certain symptoms, there is currently no known cure for multiple sclerosis.
In healthy individuals, the neuronal signal moves rapidly down the axon to the terminal buttons, where synaptic vesicles (shaped like bubbles) release neurotransmitters into the synaptic cleft. The synaptic cleft or synapse is a very small space between two neurons and is an important site where communication between neurons occurs. Once neurotransmitters are released into the synaptic cleft, they travel across the small space and bind with corresponding receptors on the dendrite of an adjacent neuron (called the postsynaptic neuron because it is after the synapse). Receptors, proteins on the cell surface where neurotransmitters attach, vary in shape, with different shapes “matching” different neurotransmitters.
Watch It
This video shows the structure and physiology of a neuron.
You can view the transcript for “2-Minute Neuroscience: The Neuron” here (opens in new window).
How does a neurotransmitter “know” which receptor to bind to? The neurotransmitter and the receptor have what is referred to as a lock-and-key relationship—specific neurotransmitters fit specific receptors similar to how a key fits a lock. The neurotransmitter floats in the synapse and binds to any receptor that it fits (see Figure 4).
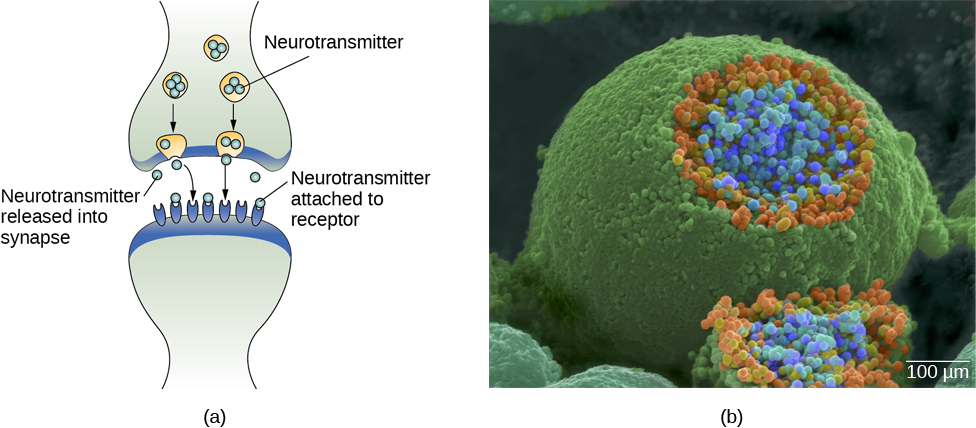
Try It
Neural Communication
SUGGESTION: As you read through the discussion below, it may help you to get out a piece of paper and summarize with bullets or numbers the process involved in neural transmission. This will help you remember the process and also help you to review the material for quizzes and exams. Alternatively, if you remember visual images better, draw out the process on a picture of a neuron, number the steps along the picture or diagram with a brief description.
To understand the details of neural communication, let’s begin at the neuronal membrane. The neuron exists in a fluid environment—it is surrounded by extracellular fluid and contains intracellular fluid (i.e., cytoplasm). The neuronal membrane keeps these two fluids separate—a critical role because the electrical signal that passes through the neuron depends on the intracellular and extracellular fluids being electrically different. This difference in charge across the membrane, called the membrane potential, provides energy for the signal.
The electrical charge of the fluids is caused by charged molecules (ions) dissolved in the fluids. The semipermeable nature of the neuronal membrane somewhat restricts the movement of these charged molecules, and, as a result, some of the charged particles tend to become more concentrated either inside or outside the cell.
Between signals, the neuron membrane’s potential is held in a state of readiness, called the resting potential. Like a rubber band stretched out and waiting to spring into action, ions line up on either side of the cell membrane, ready to rush across the membrane when the neuron goes active and the membrane opens its gates (i.e., a sodium-potassium pump that allows movement of ions across the membrane). Ions in high-concentration areas are ready to move to low-concentration areas, and positive ions are ready to move to areas with a negative charge.
In the resting state, sodium (Na+) is at higher concentrations outside the cell, so it will tend to move into the cell. Potassium (K+), on the other hand, is more concentrated inside the cell, and will tend to move out of the cell. In addition, the inside of the cell is slightly negatively charged compared to the outside. This provides an additional force on sodium, causing it to move into the cell (see Figure 5).
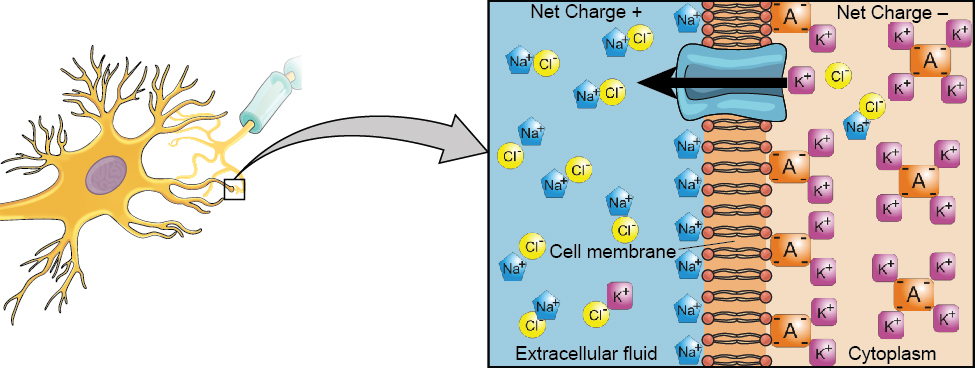
From this resting potential state, the neuron receives a signal and its state changes abruptly (Figure 6). When a neuron receives signals at the dendrites—due to neurotransmitters from an adjacent neuron binding to its receptors—small pores, or gates, open on the neuronal membrane, allowing Na+ ions, propelled by both charge and concentration differences, to move into the cell. With this influx of positive ions, the internal charge of the cell becomes more positive. If that charge reaches a certain level, called the threshold of excitation, the neuron becomes active and the action potential begins. This process of when the cell’s charge becomes positive, or less negative, is called depolarization.
Many additional pores open, causing a massive influx of Na+ ions and a huge positive spike in the membrane potential, the peak action potential. At the peak of the spike, the sodium gates close and the potassium gates open. As positively charged potassium ions leave, the cell quickly begins repolarization. At first, it hyperpolarizes, becoming slightly more negative than the resting potential, and then it levels off, returning to the resting potential.
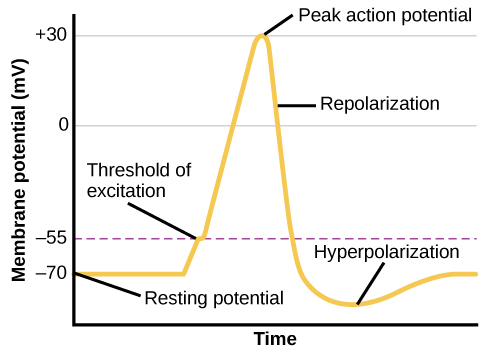
This positive spike constitutes the action potential: the electrical signal that typically moves from the cell body down the axon to the axon terminals. The electrical signal moves down the axon like a wave; at each point, some of the sodium ions that enter the cell diffuse to the next section of the axon, raising the charge past the threshold of excitation and triggering a new influx of sodium ions. The action potential moves all the way down the axon, pushing synaptic vesicles loaded with neurotransmitters towards the terminal buttons.
The action potential is an all-or-none phenomenon. In simple terms, this means that an incoming signal from another neuron is either sufficient or insufficient to reach the threshold of excitation. There is no in-between, and there is no turning off an action potential once it starts. Think of it like sending an email or a text message. You can think about sending it all you want, but the message is not sent until you hit the send button. Furthermore, once you send the message, there is no stopping it. Presynaptic neurons (those sending the signals via neurotransmitters) can either be excitatory, meaning they are encouraging the postsynaptic neuron to depolarize and “fire” the signal, or they can be inhibitory, attempting to repolarize the neuron and prevent firing of the action potential. Whether the neuron activates the action potential or not depends on the overall sum of these excitatory and inhibitory signals and whether they exceed the neuron’s threshold of excitation.
Because it is all or none, the action potential is recreated, or propagated, at its full strength at every point along the axon. Much like the lit fuse of a firecracker, it does not fade away as it travels down the axon. It is this all-or-none property that explains the fact that your brain perceives an injury to a distant body part like your toe as equally painful as one to your nose.
As noted earlier, when the action potential arrives at the terminal button, the synaptic vesicles that had been pushed down the axon by the signal wave release their neurotransmitters into the synaptic cleft. The neurotransmitters travel across the synapse and bind to receptors on the dendrites of the adjacent neuron, and the process repeats itself in the new neuron (assuming the signal is sufficiently strong to trigger an action potential). Once the signal is delivered, excess neurotransmitters in the synaptic cleft drift away, are broken down into inactive fragments by enzymes, or are reabsorbed in a process known as reuptake. Reuptake involves the neurotransmitter being pumped back into the neuron that released it, in order to clear the synapse (Figure 7) in a type of “recycling” that allows the neuron to repackage the neurotransmitters into vesicles for the next signal. Clearing the synapse serves both to provide a clear “on” and “off” state between signals and to regulate the production of neurotransmitter (full synaptic vesicles provide signals that no additional neurotransmitters need to be produced).
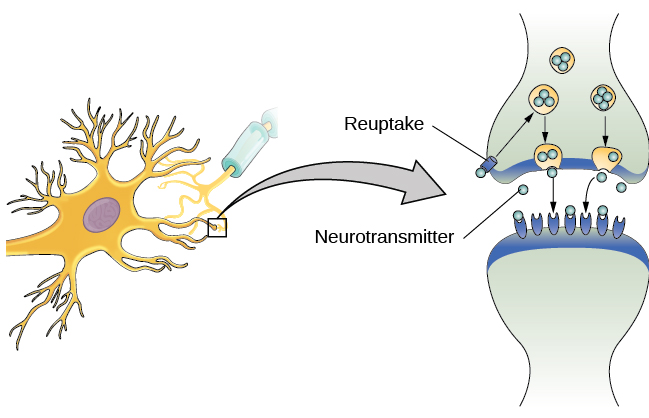
Neuronal communication is often referred to as an electrochemical event. The movement of the action potential down the length of the axon is an electrical event, and movement of the neurotransmitter across the synaptic space represents the chemical portion of the process. Understanding these processes is vital to understanding how modern psychiatry has developed medical treatments for mental disorders. For instance, some common forms of antidepressants like Zoloft, Paxil, or Celexa are classified as Selective Serotonin Reuptake Inhibitors. As this name suggests, their mechanism of action is to blockade reuptake receptors on the presynaptic neuron. This means that serotonin, a neurotransmitter, released into the synapse will not be able to be brought back into the cell and be repackaged; it will remain in the synapse for a longer period of time, creating more opportunities to bind to receptor sites on the postsynaptic neuron.
Other psychiatric drugs work by increasing the production of specific neurotransmitters in the nucleus of the neuron, blockading the receptor sites on the postsynaptic dendrite so the signal is decreased. An older antidepressant called Monoamine Oxidase Inhibitor (MAOI) inhibits the action of the enzymes in the synapse so that the neurotransmitters remain in the synapse longer and thus have a stronger signal. Other non-drug treatments, often used as “second line” interventions when medications are not effective, usually work by influencing how neurons function. One example is Transcranial Magnetic Stimulation (TMS), which involves a noninvasive pulse of magnetic energy to parts of the brain. Remember that neurons’ action potentials are triggered by ions that are positive or negative in charge (like the ends of a battery) and thus may be influenced by magnetic fields. Specific medical treatments for mental disorders will be described in the relevant modules for the mental disorders.
Neurotransmitters and Mental Disorders
The exact number of neurotransmitters in the brain is still being determined and not all of them are related to mental disorders. Remember that neurotransmitters can also be defined as excitatory (encouraging the postsynaptic neuron to fire by disrupting the resting potential) or inhibitory (calming down or restoring the resting potential across the membrane of the postsynaptic neuron and preventing an action potential). As research continues in the functioning of the brain and neurons, it is also clear that often neurotransmitter systems interact and mental disorders may be related to the functioning of several neurotransmitter systems together. The mental disorder modules will go into more details about specific neurotransmitter systems, but at this point, our best understanding is that at least five major neurotransmitter systems are involved, in various ways, with mental disorders and may have other physical effects as well (such as Parkinson’s disease as described above). These are the five neurotransmitter systems involved:
- dopamine: related to movement, memory, and as part of the brain’s pleasure or reward center
- serotonin: a neuromodulator, meaning that serotonin appears to influence the functioning of other neurotransmitter systems; serotonin is also relevant to pain, sleep, digestion, and mood
- norepinephrine: also related to mood, pain, heart rate, and blood pressure
- GABA: a major inhibitory neurotransmitter, often believed to be related to calming down or relaxation; it has links and connections to alcohol and other substances
- glutamate: an excitatory neurotransmitter, related to learning and memory and other functions
Try It
Glossary
action potential: electrical signal that moves down the neuron’s axon
agonist: drug that mimics or strengthens the effects of a neurotransmitter
all-or-none: phenomenon that incoming signal from another neuron is either sufficient or insufficient to reach the threshold of excitation
antagonist: drug that blocks or impedes the normal activity of a given neurotransmitter
axon: major extension of the soma
biological perspective: view that psychological disorders like depression and schizophrenia are associated with imbalances in one or more neurotransmitter systems
concordance rates: the probability or likelihood that if one person in a pair of people (usually twins) has a specific phenotype, that the other one will also have or will develop that trait
depolarization: when a cell’s charge becomes positive or less negative
dendrite: branch-like extension of the soma that receives incoming signals from other neurons
epigenetic influences: external factors that can change which genes in a person’s DNA may activate or may be turned off
glial cell: nervous system cell that provides physical and metabolic support to neurons, including neuronal insulation and communication, and nutrient and waste transport
hyperpolarization: when a cell’s charge becomes more negative than its resting potential
membrane potential: difference in charge across the neuronal membrane
myelin sheath: fatty substance that insulates axons
neuron: cells in the nervous system that act as interconnected information processors, which are essential for all the tasks of the nervous system
neurotransmitter: chemical messenger of the nervous system
polygenic inheritance: a trait influenced by multiple (poly) genes
psychotropic medication: drugs that treat psychiatric symptoms by restoring neurotransmitter balance
receptor: protein on the cell surface where neurotransmitters attach
resting potential: the state of readiness of a neuron membrane’s potential between signals
reuptake: neurotransmitter is pumped back into the neuron that released it
semipermeable membrane: cell membrane that allows smaller molecules or molecules without an electrical charge to pass through it, while stopping larger or highly charged molecules
soma: cell body
synapse: small gap between two neurons where communication occurs
synaptic vesicle: storage site for neurotransmitters
terminal button: axon terminal containing synaptic vesicles
threshold of excitation: level of charge in the membrane that causes the neuron to become active
Licenses & Attributions (Click to expand)
CC Licensed Content, Shared Previously
- “Biological Elements of Psychopathology.” Authored by: Anton Tolman for Lumen Learning. Provided by: Lumen Learning. License: CC BY: Attribution
- Perspectives on Psychological Disorders. Authored by: OpenStax College. Located at: http://cnx.org/contents/Sr8Ev5Og@5.52:fXTlbMdQ@5/Perspectives-on-Psychological-. License: CC BY: Attribution. License Terms: Download for free at http://cnx.org/content/col11629/latest/.
- Heritability. Provided by: Wikipedia. Located at: https://en.wikipedia.org/wiki/Heritability. License: CC BY-SA: Attribution-ShareAlike
- Cells of the Nervous System. Authored by: OpenStax College. Located at: https://openstax.org/books/psychology-2e/pages/3-2-cells-of-the-nervous-system. License: Public Domain: No Known Copyright. License Terms: Download for free at https://openstax.org/books/psychology-2e/pages/1-introduction.
- 2-Minute Neuroscience: The Neuron. Authored by: Neuroscientifically Challenged. Located at: https://www.youtube.com/watch?v=6qS83wD29PY. License: Other. License Terms: Standard YouTube License
- Cells of the Nervous System. Provided by: OpenStax College. Located at: https://openstax.org/books/psychology-2e/pages/3-2-cells-of-the-nervous-system. License: CC BY: Attribution. License Terms: Download for free at https://openstax.org/books/psychology-2e/pages/1-introduction.